According to the Nobel prize-winning biochemist Albert Szent-Györgyi, “Life is nothing but an electron looking for a place to rest.” This quote succinctly describes the importance of reduction-oxidation (redox) reactions in enabling living cells to extract energy from chemical bonds through metabolism.
This is perhaps best exemplified by the diversity of metabolic strategies performed by microbes living in the geochemical gradients found in environments such as soils, aquatic sediments, and the deep Earth.
As microbiologists study organisms from these environments, they are continually discovering electroactive microbes that move electrons in a manner distinct from the canonical electron transport chain used for aerobic respiration in the mitochondria. Many of these microbes are capable of moving electrons past the inner membrane, out to cell surface and beyond, to deposit onto extracellular materials such as oxide minerals or other cells within their environment. Recently, these microbes and the molecules they have evolved to move electrons have been coupled with both electrochemical systems and solid-state electronics paving the way for novel types of living electronics. These devices leverage the charge transporting properties of these cells and integrate them as electrical components within circuits to function as power sources, wires, and sensors just to name a few.
Early work in living electronics focused on using microbial metabolism of organic substrates as an energy source for producing electrical voltages. These studies date back to the early and middle 20th century. The first study illustrating the generation of an electrode potential from microbial metabolism came in 1911 from an English botanist Michael Cressé Potter. In this study, Potter used a galvanometer to observe the voltage generated from Escherichia coli and Saccharomyces cerevisiae metabolising sugars when exposed to a platinum electrode. The first considerations of the limits of microbial metabolism for actually powering electrical devices came in 1931 through a study by the American bacteriologist Barnett Cohen, where he constructed a bacterial battery and examined the current and voltage output which performed poorly with just the cells and the electrode. To enhance the electrochemical connection between the bacteria and the electrode he introduced chemical redox mediators to overcome the insulating barrier of the outer-membrane in these fermentative organisms. This study paved the way for a flurry of fundamental biological studies in the 1930-50s to use electrode potential to monitor bacterial growth and metabolism.
The earliest filed patent for a microbial fuel cell comes from Gilson Rohrback at TRW inc. (then Magna Corporation) depicted here in one of many proposed configurations using the sulphate-reducing bacterium Desulfovibrio desulfuricans growing on a cathode to drive electron flow to power electrical devices for remote ocean applications. Ref. US3288799
It was not until the early 1960s that the first attempts were made to apply microbes as a catalyst for energy production to power electrical devices. These efforts were driven primarily by project funding from NASA and the U.S. Navy with a goal of developing power sources for remote space and ocean environments. Early efforts were led by biologist Fred Sisler – originally at the U.S. Geological Survey then later while at General Scientific Corporation – who coined the term ‘biochemical fuel cell’ in the literature to describe the electrical device they used to test the feasibility of having sulphate-reducing bacteria power electrical devices. In parallel, work by Gilson H. Rohrback of Magna Corporation yielded the first patent covering “Biological Electrical Power Generation” also focusing on using sulphate-reducing bacteria to function as batteries to power devices deployed in the ocean. Shortly thereafter, Davis and Yarbrough at Mobil Oil Company, now ExxonMobil, modified the term to ‘microbial fuel cell’ when they described their unsuccessful efforts to use hydrocarbons as a fuel source for current generation from Norcadia also earning them an early patent for “Biochemical fuel cells, i.e. cells in which microorganisms function as catalysts.” Microbial fuel cells were even developed into a consumer product that was marketed by Allied Radio Corporation that enabled people to build their own bacterial battery to power low-powered electronics such as small electric motors, transistor radios, and a pilot lamp and featured in the February 1964 issue of Popular Electronics. While these early illustrations showed the promise of using bacteria to convert organic matter into electricity, they produced very little power limiting their practical application and intensive research in developing this technology lapsed over the next several decades.
Interest in microbial fuel cells was revived in the late 1980s, largely by Peter Bennetto of King’s College London who like Cohen in the ‘30s used redox mediators to electrically link fermentative bacteria and yeasts to an electrode. Simultaneously, environmental microbiologists were discovering microbes with the unique capabilities of producing molecules that enabled them to move electrons beyond the cell surface. Two microbes, Geobacter metallireducens and Shewanella oneidensis, were isolated from freshwater sediments in the Potomac river by Derek Lovely and in Lake Oneida by Kenneth Nealson, respectively. Both are dissimilatory metal reducing bacteria that couple the oxidation of organic matter to the reduction of metal oxides in the extracellular environment. They are able to move electrons to these far away mineral surfaces by producing cellular appendages that function as electron-carrying nanowires. Later, it was learned that these molecular components also enable these microbes to generate currents when grown on an electrode surface. These cells also produced currents at much higher per cell efficiencies than the mediator-dependent process of the early microbial fuel cells. These two environmental isolates-turned-model organisms shifted the paradigm for how to interface bacteria with electrodes and fueled a continuous stream of microbial fuel cell research ever since.
Shewanella oneidensis producing a nanowire that spans microelectrodes and connects two cells. Image courtesy of Moh El-Naggar at the University of Southern California.
In the early 2000s, the first successful application of microbial fuel cells as long-term power sources for devices was made through the development of benthic microbial fuel cells by Claire Reimers and Lenny Tender that use sediment microbes to produce electrical currents. These devices can be used to power remotely deployed environmental sensors and acoustic modems, that previously had deployment times that were limited by their batteries, for indefinite periods of time. Around the same time, the applications of microbial fuel cells were expanding beyond being purely power sources for devices into catalysts for more efficient wastewater treatment processes. This was spearheaded by early development work from Byung Hong Kim at the Korea Institute of Science and Technology and Bruce Logan at the Pennsylvania State University. By coupling microbial metabolism with electrochemical systems, treatment facilities could simultaneously degrade dissolved organics in wastewaters while also harvesting power from the stored chemical energy in this previously underutilised resource. This application of living electronics can be used to build wastewater treatment systems that can address the challenges at the ‘water-energy nexus’ to achieve the UN Sustainable Development Goals for improving “clean water and sanitation” and “climate action”. For example, recently AquaCycl, a US start-up company, illustrated how modular microbial fuel cells can more sustainably treat sugar-laden wastewater coming from a PepsiCo bottling facility, helping to reduce 110 tons of greenhouse gas emission per month from the transport and treatment of waste streams from this single facility. Devices like these are being commercially explored for onsite treatment and power recycling from diverse wastewater sources.
An engineered electron transport chain enabling Escherichia coli to produce an electrical signal in response to chemicals that bind the human estrogen receptor. With an input module that senses chemical pollutants and an output module using proteins from Shewanella oneidensis to move electrons out to the cell surface to an electrode. Atkinson and Su et al. Nature 2022
Living electronics can also be constructed that use biological components as more than power sources. Synthetic biology is enabling microbiologists to precisely program the input-output behavior of living cells similar to how electrical engineers program the behavior of electronic circuitry. Applying these techniques to engineer how microbes that are interfaced with electronics behave is paving the way to build devices with tailor made functionalities.
For example, recently living electronic sensors have been built from Escherichia coli that can detect chemical pollutants within minutes and produce an electrical signal. To generate this signal, these cells were engineered to express the proteins Shewanella oneidensis uses to move electrons to the cell surface. This enabled E. coli to move electrons to an electrode without the need for a redox mediator. To sense pollutants, in this case endocrine disrupting chemicals, these cells use engineered electron transfer proteins that function as nanoscale chemical-responsive switches to control the flow of electrons through cellular metabolism enabling the rapid response times of these sensors. By using sensor components that are proteins, these living electronic sensors can respond to organic pollutants that impact biological processes and can be customised to detect different analytes by altering the amino acid sequence of the chemical-binding components of the switches so that they respond to different targets. Often these types of organic pollutants are challenging to detect without taking water samples into a laboratory and using conventional analytical chemistry techniques like HPLC and mass spectrometry. In contrast, when embedded in hydrogels these living electronics sensors were found to function in riverine and marine waters, which indicates that they could be deployed in the environment as remote sensors to monitor for the release of pollutants in urban waterways without the need for sampling and laboratory analysis.
To build healthier and more resilient infrastructure for transporting clean water we need to develop strategies that can efficiently treat wastewater and help mitigate the release of pollutants into the environment. Living electronics are helping to solve these challenges on multiple fronts. They are improving our ability to locally treat wastewater while mitigating the carbon footprint of this essential process. They are also enabling us to monitor and electronically report when pollutants are released into the environment. The direct integration of living electronic sensors with benthic microbial fuel cells could in the future enable devices that can function as long-term deployable sensor nodes within pollutant monitoring networks. Such networks of sensors can be used to monitor and track when pollutants from human activities are introduced into the environment. This will allow for the future construction of more resilient infrastructure that can help to mitigate the spread of pollutants introduced to waterways near industrial and agricultural sites and during extreme weather events.
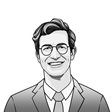
No comments yet